“Brain-sighted” mice could lead us into the world of The Matrix
A Hungarian research group has gone far beyond the benefits of retina implants by compelling the brain itself to see. We talked with the group’s leader, ERC winner Balázs Rózsa about light-sensitive nerve cells, an optogenetic revolution and a window opening on the brain which could even help restore the vision of blind people.
When one reads about a research group “who are developing an apparatus in blind people’s brains which could create a virtual reality similar to the one in the film The Matrix”, one is likely to suspect an exaggeration; however, it is quite enough to have an hour-long discussion with the leader of the research group Balázs Rózsa, to quickly realize that the world agents Neo and Smith preside in is merely a beginning. We will see mutants, fully exploited viruses, lasers guided by sound. And I have not even mentioned that at stake was 2 million Euros and by winning this sum we have been able to see into the thoughts of animals with illuminated brains as never before.
From sight to the experience of vision
In order to understand what the ERC-winning research group is working on relentlessly, it is important to get a clear picture regarding the process of vision itself. Because those of us who believe that coloured pictures are rolling continuously in our “skull cinemas” couldn’t be more wrong.
Our eyes, not unlike the lens of a camera, project the (reverse) image of the view in front of us on our retina with various light-sensitive cells producing electronic signals out of the photons. This sounds simple enough, however there are at least 19 channels that in which signals are streamed towards the brain – for example colours have their own channel, there is another one for contrasting information, what's more even a certain amount of movement recognition occurs at this stage. And things become even more complex as information passes through the thalamus, the gigantic switch-centre of the brain, through which information reaches the visual cortex where the actual experience of vision is produced.
To learn more about what could be occurring in the complicated network of nerve cells, we firstly have to turn to the case of a small fish. In their 2014 article, Jeremy Freeman and his fellow-researchers were examining the operation of the brain of one of the favourite experimental animals of biologists, the zebrafish. They did this by employing at first glance a rather unusual method. Certain nerve cells of a particular fish with fully transparent skin and skull were examined. The result was a video, (made possible thanks to a so called optogenetic method later to be discussed in the article), which provided insight into the simultaneous operation of the 80 000 brain cells of the small fish.
The video shows how the effect of the impulse reaching the eyes progresses into various parts of the brain. In the forebrain, however (in humans this occupies the greater part of the skull where the more complicated brain processes occur) the regular activity patterns are impossible to decipher, additionally there is continuous activity here even when nothing is happening in close proximity to the zebrafish.
As researchers came to examine the brains of zebrafish and later of mice and other experimental animals, it became clear that many areas of the brain show large amounts of activity when at rest, in spite of the fact that no significant impulses are arriving through the senses, certain groups of nerve cells show joint activity both in space and time. Moreover, these characteristic groups of activities also appear when we perceive something; it is as if our brain had a “language” of its own and was trying to use the words of this language (i.e. group activity patterns) in interpreting information arriving from the outside and when left alone, it would speak to itself. Were we to know this language perhaps we could feed this information directly into our brains – this is how we arrive to the world of The Matrix.
But how would we begin to acquaint ourselves with such a brilliant new language? The simplest and most convenient approach, is to first listen to users of the language express themselves in the simplest of situations, then imitate them and wait eagerly for a reaction.
Balázs Rózsa’s research group is employing exactly this method even though their experiments need somewhat more refined tools and many more mice than a practicing linguist-anthropologist.
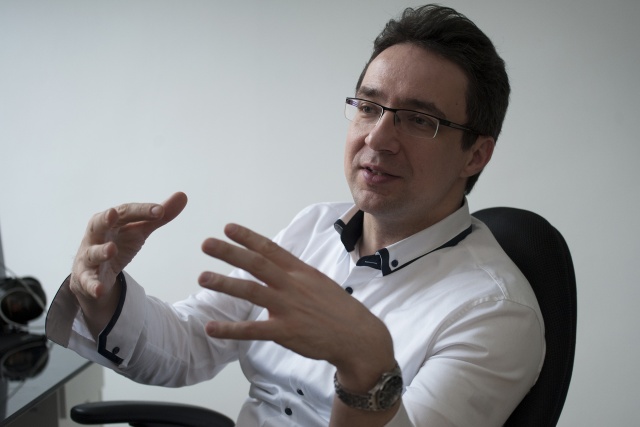
In order to be able to directly communicate with an animal’s (in our case: a mouse’s) brain we would need to employ methods that have only become available to researchers in the past few years. Earlier, researchers’ direct measuring method consisted of sticking electrodes into the brain-tissue to try and measure as well as influence the activity of certain brain cells. This method, however only allowed the activity gauging of a couple of cells and it was impossible to measure the individual functioning of several hundred or thousand nerve cells. In addition, these measurements were performed “blindly” since neither the place, nor the anatomy of those cells could be identified after the measurements, therefore a different technique was required to unravel the behaviour of larger networks.
The widely known and employed brain scanning technique fMRI is suitable to examine the activities of the brain, however this method is only suitable to measure activities of larger areas of the brain while its resolution is at least a thousand times weaker than what is needed to examine individual nerve cells.
The breakthrough came in the last decade with the emergence of optogenetics, a scientific discipline rooted in microscopy and genetics. This new scientific method helped uncover that certain proteins are able to become fluorescent, depending on the neighbouring ions’ concentration; accordingly, after being “switched on” by a change in the ion concentration, they could be seen flickering if hit by the appropriate hue of light. Other proteins are capable of the exact opposite: if hit by the appropriate shade of light, they open up a channel on the cell membrane and thus change the concentration of certain ions in the cell.
In order for these amazing findings result in an actual „conversation” with the brain a couple of minor details have to be settled, notably:
- Two kinds of protein need to be fed into the cells: one for performing measurements, the other to be activated by light.
- We must ensure that the brain of our experimental subject is in an aware state and activate it with light while our subject is performing various activities.
- We must conceive of simple situations in which we’d like to examine the code of this complicated brain language first.
- After we have “made” the brain “talk”, we must also see if it understands what we’re “
“telling” it. - Here comes The Matrix.
Benign infection, mutant mice
Let’s start with point one. Biology experts know precisely that if we are endeavouring to get foreign proteins into cells, viruses are the best possible candidates. Although it is true that viruses are selfish and strive for the contaminated cells to manufacture their own proteins exclusively, (a process that ultimately kills the cell in question). However, if we only use the protein shell of a virus to inject the genes of certain proteins, we could ingeniously modify the activities of the cell. In addition, since these viruses do not reproduce, the quantity we add can regulate precisely which cells we want to modify.
This is how we achieve that only certain, well-defined areas of a subject’s brain be light-activated and fit to emit light.
While Balázs Rózsa’s team experiments on mice, researchers abroad are also preparing experiments to be carried out on monkeys. In fact, the permissions are being prepared for special virus technologies that can pave the way for human application at first on a small scale. On an international scale, the research group of Botond Roska in Switzerland is leading the way in developing the genetic technology to restore vision.
The situation is somewhat simpler with experimental mice – one can produce genetically modified animals whose brain cells contain these proteins in the first place. This approach however is obviously (or should we say presumably) not applicable when it comes to human medicine.
A window on the brain
The next immense question is how do we get light into the brain and how do we examine it through a microscope? Fortunately for this we will not be needing the massive headjack seen in The Matrix, only a small glass-screen on the skull, right above the appropriate area of the brain. No electrodes, no wires, no complicated electrical grids on the skull, all you see is a small window on the skull of the experimental animal.
The heads of experimental mice are fixed during optical measurements, their feet, however, are free to move, while their eyes are facing monitors onto which various moving images are projected. This is how researchers create the virtual labyrinth in which the mouse is free to roam about. Its position, the direction and speed of its locomotion are all determined by the experimental equipment and based on the motion of its feet while the projected environmental features also change according to this input.
Consequently, the mouse genuinely believes it is wandering in a labyrinth while activity patterns are generated in the nerve cell networks of its brain. This is what researchers are observing through the screen created above the visual cortex with the assistance of a microscope connected to a system of lasers and thanks to the services of proteins capable of light-emittance infused into the nerve-cells earlier.
Through a reward and punishment scheme the mouse is taught to avoid certain areas while others it is trained to seek out. Once it is comfortably moving around in the maze projected on the monitor, the activities of its brain cells is measured with a microscope connected with a system of lasers in a given part of its visual cortex. Thus, we have reached the simple situations mentioned at the beginning of our article, suitable for recording the words of the interior “language” of the brain.
Confined brain movements, sound-controlled lasers
Unfortunately, it is not enough to look into the brain with a super-microscope in order to read the activity patterns of the neural network since the living and functioning brain keeps moving around, no matter how hard we attempt to secure the skull. Albeit not a great deal, but just enough to make an offshoot of a nerve cell disappear in a fraction of a second.
One of the great results of the research team of Balázs Rózsa’s is the invention of a sound controlled laser system (the name acousto-optic refers exactly to this) that can scan various elements – offshoots of cells, precisely specified small volumes – as well as correct the tiny movements of the brain mentioned above. With these methods it is possible to achieve that the microscope really follows the behaviour of the nerve cell network while discarding false values that are common when the sample is displaced between two measurements.
So far we have spoken little about the obvious fact that the brain is a three-dimensional object. This could cause serious problems in observation; luckily, neither the light signals appearing in experiments, nor the special excitational light signals, the so-called two-photon impulses, get overmuch absorbed in the pulpous matter of the brain. Thus, using the third dimension of scanning, one can measure the activity of neural networks in the full width of the cerebral cortex with speeds above a kilohertz that is a thousand measurements per second.
In addition, they can do all this within large cubic volumes since Balázs Rózsa and his colleagues have realised that once they have made a three-dimensional picture of a three-dimensional object, they do not have to deal with one of the most intrinsic limitations of traditional optic systems, i.e. correcting the picture for a two-dimensional plane. (The same principle applies to pictures taken by cameras to ensure that the entire picture is sharp, not merely the center.) Thus, a microscope is in the making which can take a picture of a volume substantially larger than before and could be suited later to examining human vision.
Recently the team applied for a Hungarian innovation fund, to support these efforts and among the plans is the start of a new, substantial collaboration so that this technology could be utilized in human medicine and therapy.
What are you looking at blind mouse?
Once our mouse has wandered around the maze for long enough it is time for the second, even more exciting part of our experiment. The researchers gradually dim the monitors and steadily begin to activate the examined nerve cells with the recorded data. The result is amazing:
a mouse can find its bearings in this (twofold) virtual maze as well!
This all sounds great, but what happens if we try to introduce another mouse’s experience of vision into the brain of our experimental animal? After all, this would be our ultimate goal: to make the brain accept information that originates from a different source.
Fortunately, the brain itself comes to our rescue. It was proven in the 1970’s that the brain can learn how to move a robot arm through a built in electrode within the brain tissue. Today, this has become a reality as there are human artificial limbs functioning according to this very principle. Surprisingly, it turned out that an electrode doesn’t have to be placed in the area responsible for moving the limbs; other brain areas can also learn to perform this task, albeit somewhat slower.
Thus, we do not have to worry how we hit the exact same areas of the brains on a cellular level where the visual cortex information of the other mouse comes from (how on earth could we considering that no two brains are alike). The brain can "understand" and learn upon the received data even if the data is taken from somewhere remotely different.
The Matrix is coming – but when exactly?
When I asked him about the long route an experiment goes through from mice to men, Balázs Rózsa remarked several times that their present project was in no way aimed at developing medical procedures, they were doing basic research. Even so, he was very keen to discuss future possibilities.
They are keeping it simple and are not planning on surpassing the recognition of simple patterns, contrasts, corners (i.e. the labyrinth experiment), however, they are looking forward to examining mammals with more developed brains (e.g. ferrets). These experiments may make it possible one day to restore the simple experience of vision to blind people whose blindness was not induced by an injury to their cerebral cortex.
The difficulty is caused precisely by the attributes of the present method namely strict stationariness: the head is secured with a microscope fixed on it with a small window on the skull. Other research groups have started to test mobile implants that go directly in the skull which could provide practical results much sooner including minimal depth perception. The microscopic system developed by Balázs Rózsa and his research team will be used to simulate as well as perfect the activities of implants, and considering how quickly the miniaturization technology is developing even a portable microscope is conceivable, one that could worn like a “hat”.
If we reconsider the case of the electrode that can be inserted anywhere and the incredible flexibility of the brain Balázs Rózsa’s and his colleagues’ method suggests another promising prospect. Because it is possible that as a last resort some other areas of the brain can also be taught “to see”. Of course this begs the question: how does this affect the original function of the target area, i.e. what are we prone to lose by such an intervention?
However, let us now return to reality and say a few words about why the ERC project of Balázs Rózsa and his colleagues received a special, high priority status.
A universal tool in brain research
The optogenetic revolution that has been going on for the past ten years has reached a level where researchers are able to examine great networks of nerve cells in living tissue. The project of Balázs Rózsa and his colleagues provides a new tool in this
- a totally new microscope construction that is capable of using 3D technology
- greatly increases the amount of cells that can be measured and activated – it used to be 10-30 cells employing traditional methods, today this number is around half a million per second
- the measurable volume has also greatly increased: today’s device is capable of examining volumes of 4x4x1 mm in high resolution
- it can optically and algorithmically compensate for the inner movements of living tissue
- it vastly increases the speed of measurement; in the zebrafish experiment mentioned earlier two shots were taken every second, while the Hungarian researchers’ equipment is able to keep up with the frame rate of video footages.
A 3D microscope connected to a system of lasers such as the one in question may very well occupy a central role in many brain research projects in the future; consequently it is by no means coincidental that it enjoys such an eminent status among other projects subsidized by the ERC.
The project consists of physicists Gergő Katona, Gergely Szalay, engineers Pál Maák, Máté Veress, Ádám Krúdy, József Mikó and Zoltán Farkas and biologists Balázs Chiovini, Linda Judák and Zoltán Szadai. Among the many international collaborations needed for such a project to take off the ground, moreover succeed we should definitely highlight the ongoing collaboration with Swiss researchers Botond Roska and Dániel Hillier.